Oxidizing agents are required to oxidize organic molecules. In organic lab, you never used dioxygen as an oxidizing agent.
It is difficult to limit the extent of oxidation using dioxygen. In addition, side reactions are likely given the nature of
the reactive oxygen reduction products. (The mechanisms of combustion reactions of organic molecules with dioxygen to produce
carbon dioxide and water are very complicated.)
INITIATION
CH4 --> CH3.
+ H .
O2 --> O3.
PROPAGATION
CH4 + H . -->
CH3. + H2
CH4 + HO . -->
CH3. + H2O
CH3. + O .
--> CH2O + H.
CH2O + HO . ->
CHO. + H2O
CH2O + H . ->
CHO. + H2
CHO. --> CO +
H.
CO + HO . -> CO2
+ H.
BRANCHING
H. + O2
--> HO. + O.
TERMINATION
H . + R.
+ M -> RH + M*
In organic lab, other oxidizing agents are often used, including permanganate
and chromate.
Figure: permanganate
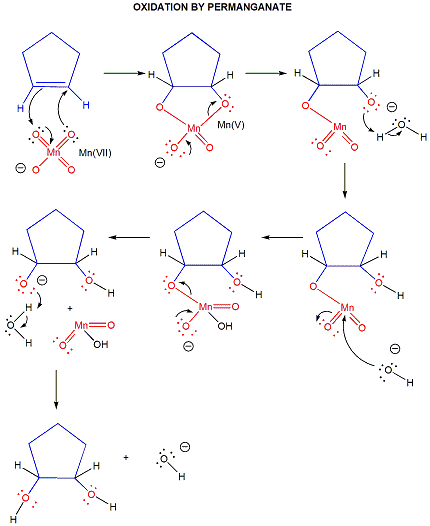
Figure: chromate
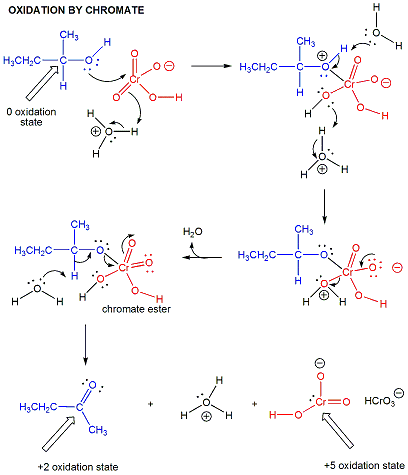
Oxygen can often be inserted into a molecule in a nonoxidative process by hydration of
an alkene to an alcohol (a readily reversible reaction), which could then be oxidized to either an aldehyde/ketone or carboxylic
acid using an appropriate oxidizing agent.
Most biological oxidation reactions (such as those found in glycolysis, Kreb Cycle, and fatty acid oxidation)
do not use dioxygen as the immediate oxidizing agent. Rather they use nicotinamide adeninine dinucleotide (NAD+)
or flavin adenine dinucleotide (FAD) as oxidizing agents, which get reduced. Enzymes that uses these oxidizing agents
are usally called dehydrogenases. Dioxygen can also be used to introduce oxygen atoms into biological molecules
in oxidative reactions. Enzymes that introduce one oxygen atom of dioxygen into a molecule (and the other oxygen into
water) are called monooxygenases. Those that introduce both atoms of dioxygen into a substrate
are called dioxygenases. These oxygenases are not usually used to oxidize organic molecules
for energy production. Rather they introduce O atoms for other reasons, including increasing the solubility of nonpolar aromatics
to facilitate secretion, and to produce new molecular species which have different biological activities. Finally, biological
molecules can be oxidized by dioxygen in which no atoms of oxygen are added to the substrate. Rather, electrons lost from
the oxidized substrate are passed via intermediate electron carriers to dioxygen , which get reduced to superoxide (if
one electron is added), hydrogen peroxide (if two electrons are added) or water (if 4 electrons are added). These enzymes
are called oxidases. (Note: The letters oxi-
or oxygen- are used in all the enzymes that use dioxygen
as the oxidizing agent.)
In this chapter section, we will discuss biological oxidation reactions. Most introductory biochemistry
texts don't approach oxidation reactions in one cohesive chapter. Probably because of that, when I was learning
biochemistry, I found the presentation of these different enzymes involved in redox reactions to be very confusing.
Hopefully this section will alleviate that problem. First the chemistry of NAD+ and FAD will be discussed.
Then the enzymes using dioxygen in oxidative reactions (monooxygenases, dioxygenases, and oxidases) will be explored.
THE CHEMISTRY OF NAD+ AND FAD
NAD+ is a derivative of nicotinic acid or nicotinamide.
Figure: NAD+ is a derivative of nicotinic acid or nicotinamide.
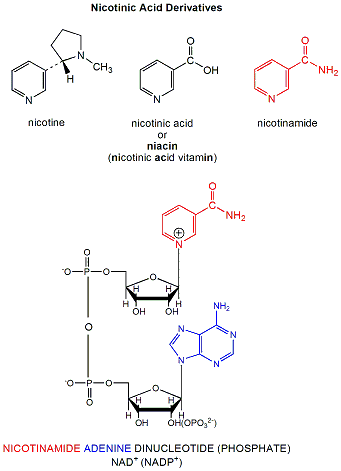
It and its reduction product, NADH, exists in the cells as interconvertible members of a pool whose total
concentration does not vary significantly with time. Hence, if carbohydrates and lipds are being oxidized by NAD+
to produce energy in the form of ATP, levels of NAD+ would begin to fall as NADH rises. A mechanism must be be
present to regenerate NAD+ from NADH if oxidation is to continue. As we will see later, this happens in the muscle
under anaerobic conditions (if dioxygen is lacking as when you are running a 100 or 200 m race, or if you are being chased
by a saber-toothed tiger) when pyruvate + NADH react to form lactate + NAD+.

Under aerobic conditions (sufficient dioxygen available), NADH is reoxidized in the mitochondria by electron
transport through a variety of mobile electron carriers, which pass electrons to dioxygen (using the enzyme complex cytochrome
C oxidase) to form water.
NAD+/NADH can undergo two electron redox steps, in which a hydride
is transferred from an organic molecule to the NAD+, with the electrons flowing to the positively charged nitrogen
of NAD+ which serves as an electron sink. NADH does not react well with dioxgyen, since
single electron transfers to/from NAD+/NADH produce free radical species which can not be stabilized effectively.
All NAD+/NADH reactions in the body involve 2 electron hydride transfers.
Figure: All NAD+/NADH reactions in the body involve 2 electron hydride transfers
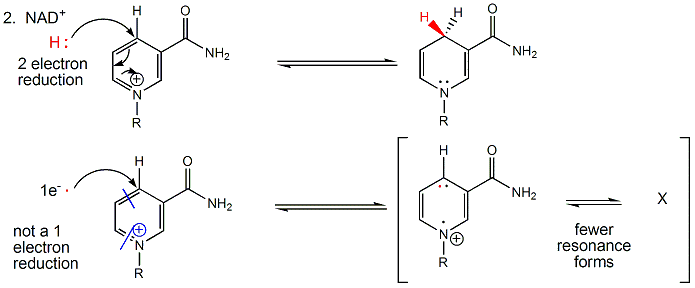
FAD (or flavin mononucleotide-FMN) and its reduction product, FADH2,
are derivatives of riboflavin.
Figure: derivatives of riboflavin
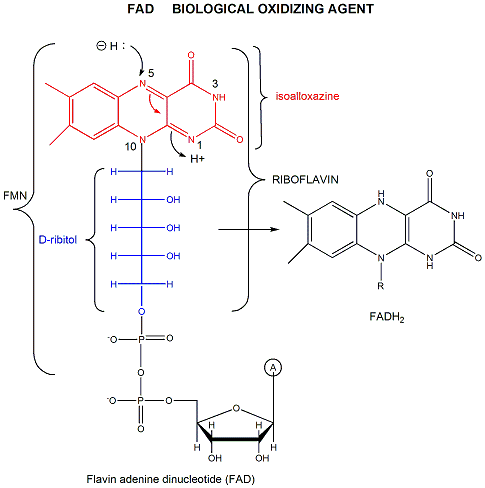
FAD/FADH2 differ from NAD+/NADH since they are bound tightly
(Kd approx 10-7 - 10-11 M) to enyzmes which use them. This is because FADH2
is susceptible to reaction with dioxygen, since FAD/FADH2 can form stable free radicals arising from single electron
transfers. FAD/FADH2 can undergo 1 OR 2 electrons transfers.
Figure: FAD/FADH2 can undergo 1 OR 2 electrons transfers
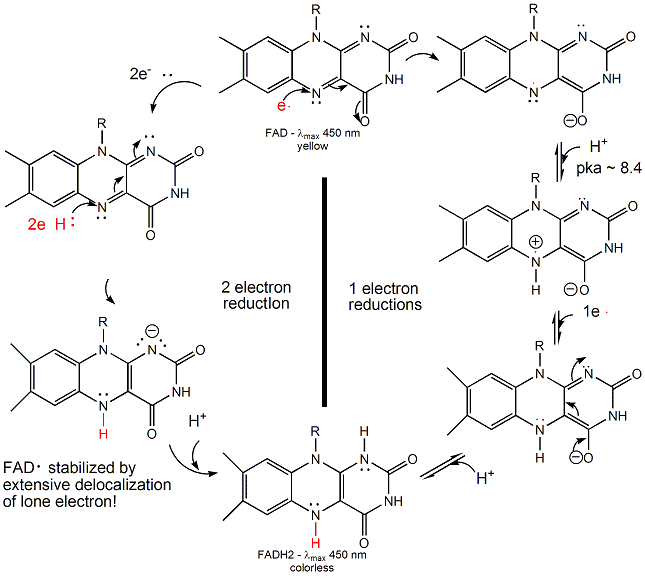
FAD/FADH2 are tightly bound to enzymes so as to control the nature of the oxidizing/reducing
agent that interact with them. (i.e. so dioxygen in the cell won't react with them in the cytoplasm.) If bound FAD is used
to oxidize a substrate, the enzyme would be inactive in any further catalytic steps unless the bound FADH2 is reoxidized
by another oxidizing agent.
DEHYDROGENASES
These enzymes usually involve NAD+/NADH and are named for the substrate that is oxidized by
NAD+. For instance in the reaction:
pyruvate + NADH <===> lactate + NAD+
which is used to regenerate NAD+ under anerobic conditions, the enzyme is named lactate dehydrogenase.
As in acid/base reactions, when the preferred direction for the reaction (from a DGo
perspective) is from stronger acid to weaker (conjugate) acid, the preferred direction for a redox reaction is in the direction
from strong to weak oxidizing/reducing agents. This can easily be determined from charts of standard reduction potentials,
and using the equation: DGo = -nFDEo,
- where F is the Faraday constant (96,494 Coulombs/mol e- = 96, 494 J/(V.mol)
= 23.06 kcal/(V.mol) . One Faraday is the charge per one mol of electrons).
- and DEo, the standard EMF or standard
cell potential (total voltage at standard state conditions), which can be determined by adding the standard reduction
potentials (Eo) for the two appropriate
half-reactions, after reversing the equation for the half-reaction that represents the oxidation.
When n=2 (number of electrons) which is common for oxidations of organic molecules,
DGo (kcal/mol) = - 46.12DEo or for government work DGo
(kcal/mol) = - 50DEo
Notice when DEo > 0, DGo < 0, the reaction as written is favored under standard conditions.
Note in the table below that many of the half reactions involve protons. For biological reactions involving free protons,
the standard state concentration for the protons are not 1 M as for other solutes in solution, but defined to be the hydronium
ion concentration at pH 7.0. The DEo and DGo values for the reactions involving hydrogen ions at a standard state of
pH 7.0 are usually written as DEo' and DGo'
Table: Standard Reduction Potential Table (E0'), 25oC
oxidant |
reductant |
n (electrons) |
EoŽ (volts) |
Acetate + carbon dioxide |
pyruvate |
2 |
-0.70 |
succinate + CO2 + 2H+ |
a-ketoglutarate + H2O |
2 |
-0.67 |
acetate |
acetaldehyde |
2 |
-0.60 |
glycerate-3-P |
glyceraldehyde-3-P
+ H2O |
2 |
-0.55 |
O2 |
O2-
|
1 |
-0.45 |
ferredoxin
(ox) |
ferredoxin
(red) |
1 |
-0.43 |
Carbon dioxide |
formate |
2 |
-0.42 |
2H+ |
H2
|
2 |
-0.42 |
a-ketoglutarate + CO2 + 2H+ |
isocitrate |
2 |
-0.38 |
acetoacetate |
b-hydroxybutyrate |
2 |
-0.35 |
Cystine |
cysteine |
2 |
-0.34 |
Pyruvate + CO2 |
malate |
2 |
-0.33 |
NAD+ + 2H+ |
NADH + H+ |
2 |
-0.32 |
NADP+ + 2H+ |
NADPH + H+ |
2 |
-0.32 |
FMN (enzyme bound) |
FMNH2 |
2 |
-0.30 |
Lipoic acid, ox |
Lipoic acid, red |
2 |
-0.29 |
1,3 bisphosphoglycerate + 2H+ |
glyceraldehyde-3-P + Pi |
2 |
-0.29 |
Glutathione, ox |
red |
2 |
-0.23 |
FAD (free) + 2H+ |
FADH2 |
2 |
-0.22 |
Acetaldehyde + 2H+ |
ethanol |
2 |
-0.20 |
Pyruvate + 2H+ |
lactate |
2 |
-0.19 |
Oxalacetate + 2H+ |
malate |
2 |
-0.17 |
a-ketoglutarate + NH4+ |
glutamate |
2 |
-0.14 |
FAD + 2H+ (bound) |
FADH2 (bound) |
2 |
0.003-0.09 |
Methylene blue, ox |
Methylene blue, red |
2 |
0.01 |
Fumarate + 2H+ |
succinate |
2 |
0.03 |
CoQ (Ubiquinone - UQ + H+ |
UQH. |
1 |
0.031 |
UQ + 2H+ |
UQH2 |
2 |
0.06 |
Dehydroascorbic acid |
ascorbic acid |
2 |
0.06 |
Ubiquinone; ox |
red |
2 |
0.10 |
Cytochrome b2; Fe3+ |
Cytochrome b2; Fe2+ |
1 |
0.12 |
Cytochrome c1; Fe3+ |
Cytochrome c1; Fe2+ |
1 |
0.22 |
Cytochrome c; Fe3+ |
Cytochrome c; Fe2+ |
1 |
0.25 |
Cytochrome a; Fe3+ |
Cytochrome a; Fe2+ |
1 |
0.29 |
1/2 O2 + H2O |
H2O2
|
2 |
0.30 |
Cytochrome a3; Fe3+ |
Cytochrome a3;
Fe2+ |
1 |
0.35 |
Ferricyanide |
ferrocyanide |
2 |
0.36 |
Cytochrome f; Fe3+ |
Cytochrome f; Fe2+ |
1 |
0.37 |
Nitrate |
nitrite |
1 |
0.42 |
Photosystem P700 |
. |
. |
0.43 |
Fe3+ |
Fe2+ |
1 |
0.77 |
1/2 O2 + 2H+ |
H2O |
2 |
0.816 |
The mechanism for the oxidation of a substrate by NAD + involves concerted hydride transfer
to one face of NAD+.
Consider for example the oxidation of ethanol to acetaldehyde by alcohol dehydrogenase.
Figure: oxidation of ethanol to acetaldehyde by alcohol dehydrogenase
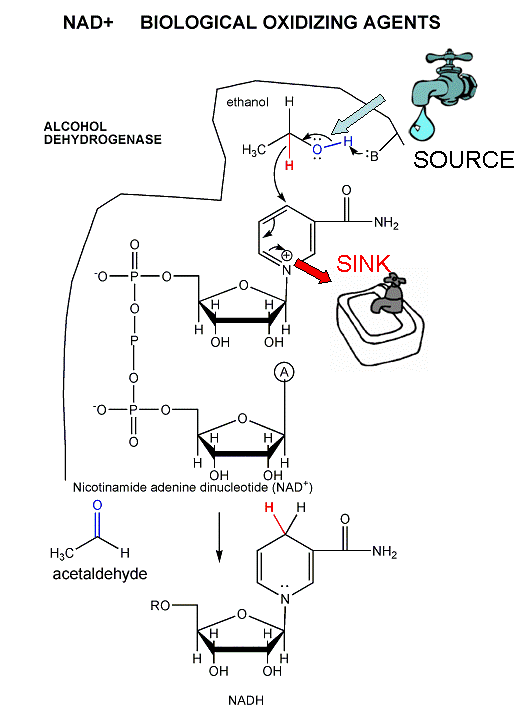
For substrates like ethanol that loses a hydride from a methylene carbon atom that has two H's, only one
of the H's is lost (either the proR or proS) from the prochiral center. (Remember the reaction of prochiral glycerol to give phospholipids.)
Figure: STEREOCHEMISTRY OF NAD+/NADH REDOX REACTIONS WITH ALCOHOL DEHYDROGENASE
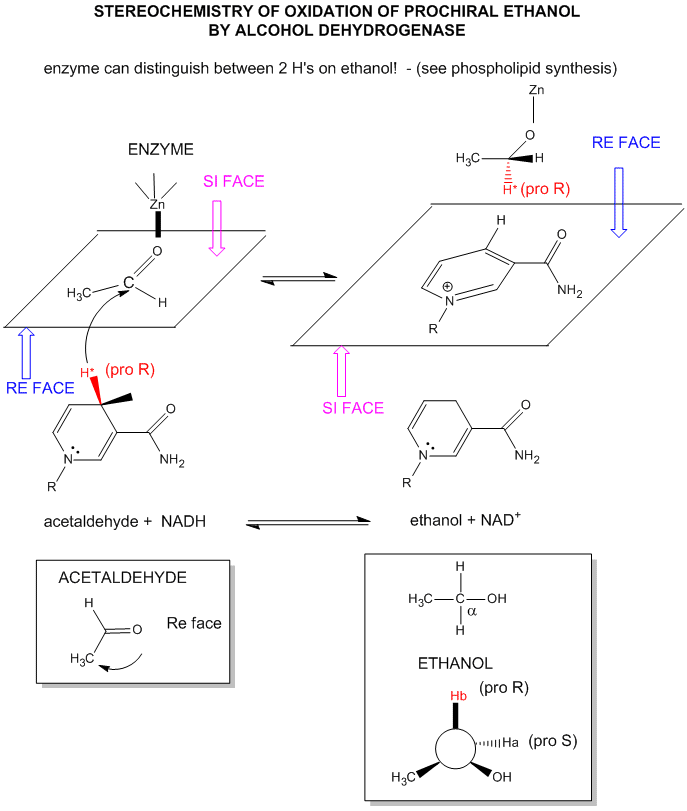
FAD has a more positive reduction potential than NAD+ so it is used for more "demanding" oxidation
reactions, such as dehydrogenation of a C-C bond to form an alkene. You will notice on standard reduction potential tables
that the potential of FAD is often listed several times and depends on the enzyme. This is because the FAD is tightly bound
to the enzyme so its tendency to acquire electrons depends on its environment, in much the same fashion as the pKa of an amino
acid side chain (which reflects is tendency to release protons) is affected by the environment of the amino acid side chain
in the protein. The standard reduction potential for flavin enzymes varies from -465 mv to + 149 mV. Compare this to
the reduction potential of free FAD/FADH2, which in aqueous solution is -208 mV. The standard reduction potential of
the flavin in D-amino acid oxidase, a flavoprotein, is about 0.0 V. Remember, the more positive the standard reduction
potential, the more likely the reactant will be reduced and hence act as an oxidizing agent. Hence the FAD in
D-amino acid oxidase is a better oxidizing agent than free FAD. A reason for this should be clear. The Kd for
binding of FAD to the enzyme is 10-7M compared to the Kd for binding of FADH2, which is 10-14M.
By gaining electrons, the flavin binds more tightly, which preferentially stabilizes the bound FADH2 compared to
the bound FAD. This shifts the equilibrium of FAD <=> FADH2 to the right, making the bound FAD a stronger
oxidizing agent.
Figure: FAD AND OXIDATIONS: MECHANISM
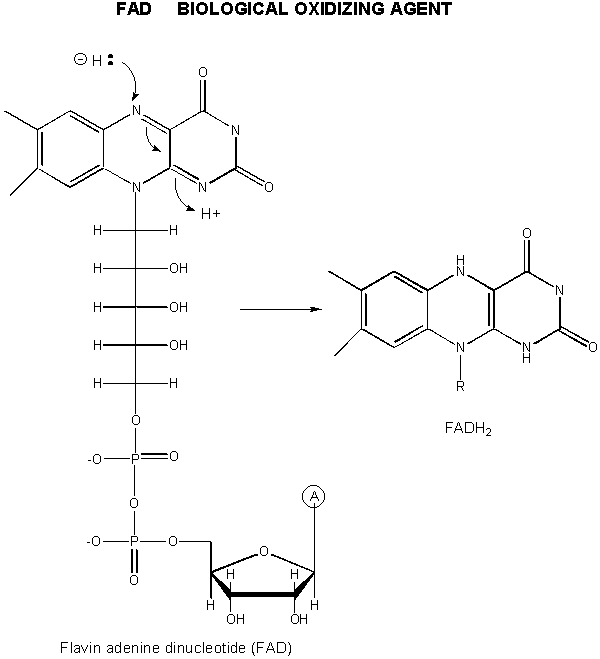
Chime Model: Flavin dehydrogenase
New Role for NAD
Investigations of aging in many organisms have shown that a plethora of proteins seem to be involved.
A recent studied show that overexpressing the yeast Sir2 (silence information regulator) caused an increase in lifespan.
The enzyme product of this gene appear to be involved in inhibition, through covalent modification of chromatin and associated
gene silencing, of genetic recombination. Investigators have shown that this protein is a NAD+-dependent
histone deacetylase. Sir2 is a member of a large family of conserved protein deacetylases called sirtuins. Accompanying
the deacetylase activity is the conversion of NAD+ to nicotinamide and acetyl-ADP ribose. Since the
enzyme uses NAD+, its activity would be related to the metabolic state of the cell. It was thought that high
levels of NAD+ would be present when metabolism was slow, and these high levels of NAD+, through its
activation of Sir2, would lead to the silencing of genes and longer lifespan. Lack of recombination would decrease DNA
damage. Alternatively, low levels of nicotinamide (a product of Sir2) could minimize inhibition of Sir2 and raises its
activity. Recently, however, Anderson et al. reported that in yeast, caloric restriction actually reduces NAD+,
and that the activity of Sir2 does not depend on the ratio of NAD+/nicotinamidel, suggesting that Sir2 is activated
(during caloric restriction) by other molecules.
It has long been know that caloric restriction increases lifespans in a variety of animals. This provides
a mechanism that would account for that. When glucose levels in culture media are restricted, the life span of
the yeast increases, as measured by an increase in the reproductive life span of the organism. This effect is only observed
if a functional Sir2 gene is present. Deacetylation of histone proteins by Sir2, would lead to an increased
positive charge on the histones (protein components of the nucleosome), which presumably increases the binding affinity of
histones (in nucleosomes) for DNA. This could reduce gene expression (i.e. induce silencing) since the nucleosomal
DNA would be less available for transcription. Surprisingly, a precursor of cellular NAD+, nicotinamide,
when given to cells to increase NAD+ levels which should silence gene expression had the opposite effect.
The effect of Sir2 on life span has also been observed in a more complex organism, C. Elegans (round worm).
Another activity of Sir2 in yeast has been noted by Aguilaniu et al. When yeast cells divide, daughter cells do not
inherit small DNA circles which code for ribosomal RNA. The concentration of these circles of DNA increase with age,
and are associated with senescence of the maternal cells. Cells without Sir2 have more of these circles. Another
feature of aging cells is the accumulation of oxidized proteins (as evidenced by an increase in carbonyl groups). In
a fashion similar to rDNA circles, oxidatively-modified proteins are not inherited by daughter cells except in mutant yeast
cells lacking Sir2.
Since increasing levels of Sir2 increase lifespan, ligands that bind to existing Sir2 and activate its deacetylase activity
might also increase lifespan. Howitz et al. screened libraries of compounds for such ligands and found one exceptionally
good one that activated the enzyme 13X: resveratrol, a polyphenol found in red wine.
Figure: resveratrol
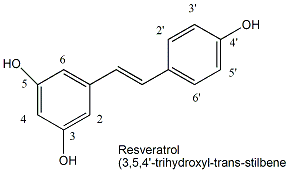
Studies have shown the people who drink moderate amounts of red wine may have lower risk for certain disease (for example
some cancers and cardiovascular disease) and longer life spans. When given to yeast, resveratrol increased their life
span by 70% in a process that required Sir2. Mutant strains lacking Sir 2 did not accrue the effect. Whether resveratrol
levels found in wine are sufficient to promote this effect in humans is unclear. Wood et al. have recently extended
the effects of resveratrol on activation of Sir2 and increased life-span to more complex organism - C. elegans and
Drosophila melanogaster.
Hydrogenases (not dehydrogenases): A break from oxidation reactions
Our world desperately needs an energy efficient way to produce H2 for energy production without producing waste
pollutants. Catalytic cracking of molecules and newly developed fuel cells offer two possibilities. Wouldn't it be great
if water could be used for H2 production (without the use of electrolysis) or expensive metal catalysts?
Nature may show the way. Bacteria (even E. Coli found in our GI system) can use simple metals like iron to produce H2
from water (which can be used by the organism as an energy source). The enzymes that catalyze hydrogen production are
hydrogenases (not dehydrogenses).
Crystal structures of hydrogenases show them to be unique among metal-containing enzymes. They contain two metals bonded
to each other. The metal centers can either be both iron or one each of iron and nickel. The ligands interacting
with the metals are two classical metabolic poisons, carbon monoxide and cyanide. Passages for flow of electrons and
H2 connect the buried metals and the remaining enzymes. The metals are also bound to sulfhydryl groups of cysteine
side chains. It appears that two electrons are added to a single proton making a hydride anion which accepts a proton
to form H2. In the two Fe hydrogenases, the geometry of the coordinating ligands distorts the bond
between the two iron centers, leading to irons with different oxidation numbers. Electrons appear to flow from one center
to the other, as does carbon monoxide as well. Ultimately, hydrogenases or small inorganic mimetics of the active site
could be coated on electrodes and used to general H2 when placed in water in electrolytic experiments.
MONOOXYGENASES
Examples of monooxygenases include the monoamine oxidases, which hydroxylate amino acids like Trp and
Tyr to form 5-hydroxytrytophan and 3-4-dihydroxyphenylalanine or dopa, respectively.
These latter two substances can be decarboxylated to form the neurotransmitters 5 hydroxytryptamine (5HT or serotonin) and
dopamine. The latter can be hydroxylated again to form norepinhephrine, and subsequently methylated to form epinephrine.
LSD and amphetamine are anaologs of serotonin and dopamine, respectively.
Figure: DIAGRAM: TRP AND TYR CHEMISTRY
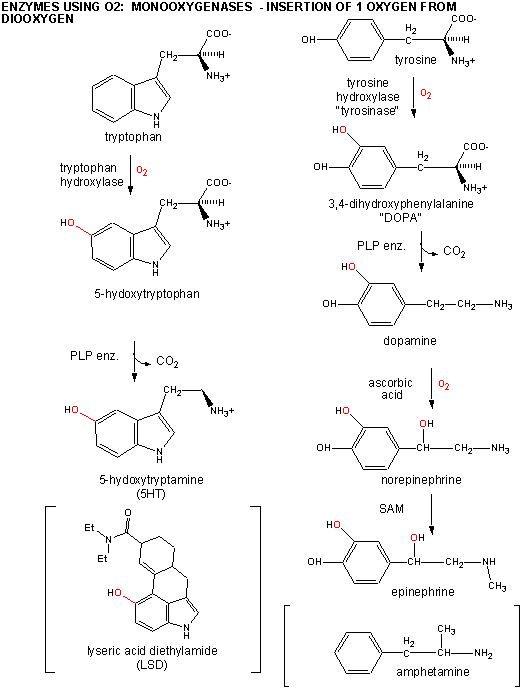
Since these monooxygenases use dioxygen, you might expect that the enzymes would use the motiffs described
in the previous section to facilitate its reaction with dioxygen. In fact, the enzyme contains a metal ion (Fe2+)
bound to a heme in the protein. In addition, the reduction products of dioxygen that are eventually used to hydroxylate
the substrate stay bound to the enzyme.
Figure: MONOOXYGENASES: POSSIBLE MECHANISM
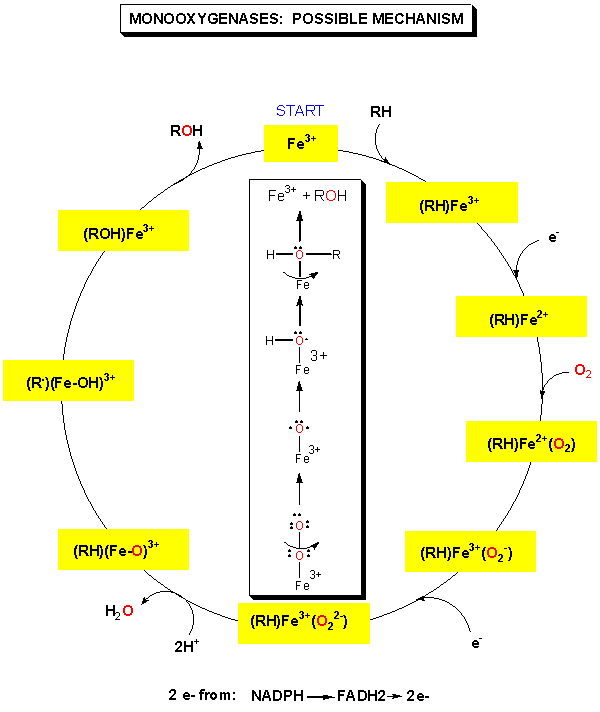
An important class of monoxygenases are called cytochromes P450's.
They represent a class of similar enzymes that each contain a heme. Instead of reversibly carrying dioxygen as does
the heme in myoglobin and hemoglobin, the P450 heme activates dioxgyen for hydroxylation reactions involving aromatic, nonpolar
substrates. Hydroxylation of these substrates increases their solubility which facilitates their elimination from the body.
Epoxide intermediates or products are often produced, which can open up through nucleophilic attack using an alcohol (sugar
derivative) or an amine (such as in nucleotide bases in DNA) to form large adducts. Hence cytochrome P450 can actually activate
aromatic substrates to become carcinogens.
Figure: CYTOCHROME P450'S: POSSIBLE MECHANISM
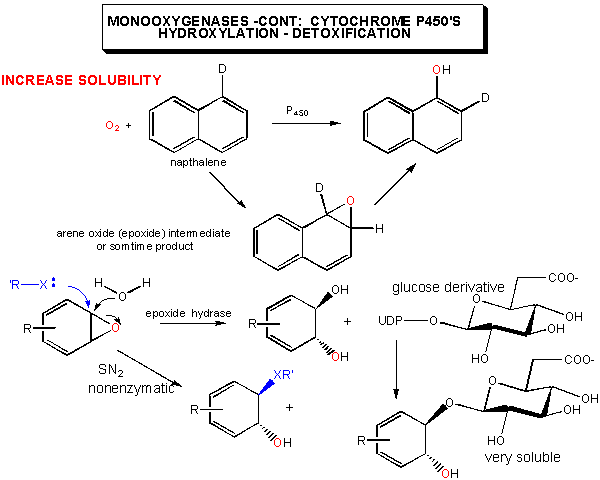
Figure: CYTOCHROME P450'S: ACTIVATION OF CARCINOGENS
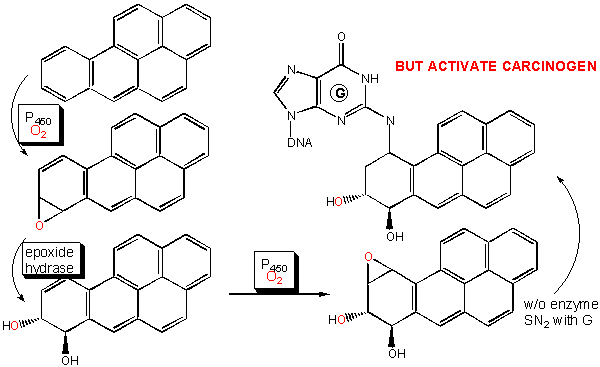
The cytochrome P450s family of genes/proteins are inducible on exposure to nonpolar aromatic molecules
such as dioxin. These nonpolar molecules can enter the cytoplasm where they bind to the arylhydrocarbon receptor (AhR) which
is bound to a heat shock protein, Hsp90. Upon binding of dioxin, TCDD, for example, the AhR.TCCD complex
dissociates from Hsp90, and migrates to the nucleus where it binds a protein called Amt. The AhR-Amt complex serves
as an enhancer/transcription factor, facilitating the transcription of the cytochrome P450 genes.
Figure: Cytochrome P450s inducible on exposure to nonpolar aromatic molecules such as dioxin.
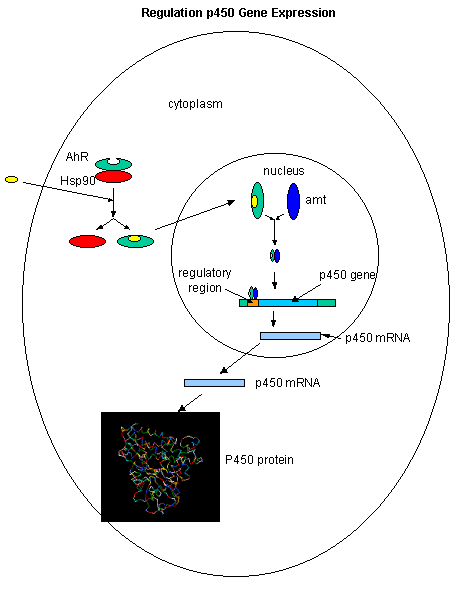
Dioxin has been shown to affect estrogen-mediated activities. Estrogens, small hydrophobic hormones derived from
cholesterol, enter cell and bind to cytoplasmic estrogen receptors, which then dimerize and bind to the estrogen response
element (ERE), initiating transcription. Dioxin binds to the arylhydrocarbon receptor (AhR). This complex can
then bind to Arnt - arylhydrocarbon nuclear transporter (a chaperone). This ternary complex can then bind to
the xenobiotic response element (XRE). Ahr and ARnt contain a basic helix-loop-helix motif which mediate their interaction
with DNA. Upon binding of the complex, detoxification genes are activated. Ohtake et al. found that the dioxin-Ahr-Arnt
complex can bind to the estrogen receptor, which can then lead to activation of the ERE in the absence of estrogen.
However, if estrogen is present, inhibition of gene expression from ERE is observed. Dioxins can be potent disregulators
of estrogen-induced gene expression. Such changes in esterogen activity could help to explain the pro- and inhibitory
effects of dioxin on estrogen-mediated cellular responses and possible effects of dioxin on the immune system and on cancer
development.
Recently, "snapshots" of P450cam, the cytochrome P450 that hydroxylates camphor, have been
taken in various stages of catalysis. This enzyme is "the biological equivalent of a blowtorch: P450 enzymes catalyze
the stereospecific hydroxylation of nonactivated hydrocarbons at physiological temperature - a reaction that, uncatalyzed,
requires extremely high temperatures to proceed, even nonspecifically". Crystal structures of normal intermediates
(such as the dioxygen-bound intermediate, could not be determined with traditional techniques since the complex had a half-life
of 10 minutes at 4oC. This problem was solved by using crystals frozen at -185oC, and by using
short wavelength X-rays, which did not cause the reaction to be driven forward. Short-time X-ray data was collected,
then substrate added to push the reaction to the next intermediate, before new structural data was obtained.
Figure: Reaction Pathyway of P450cam
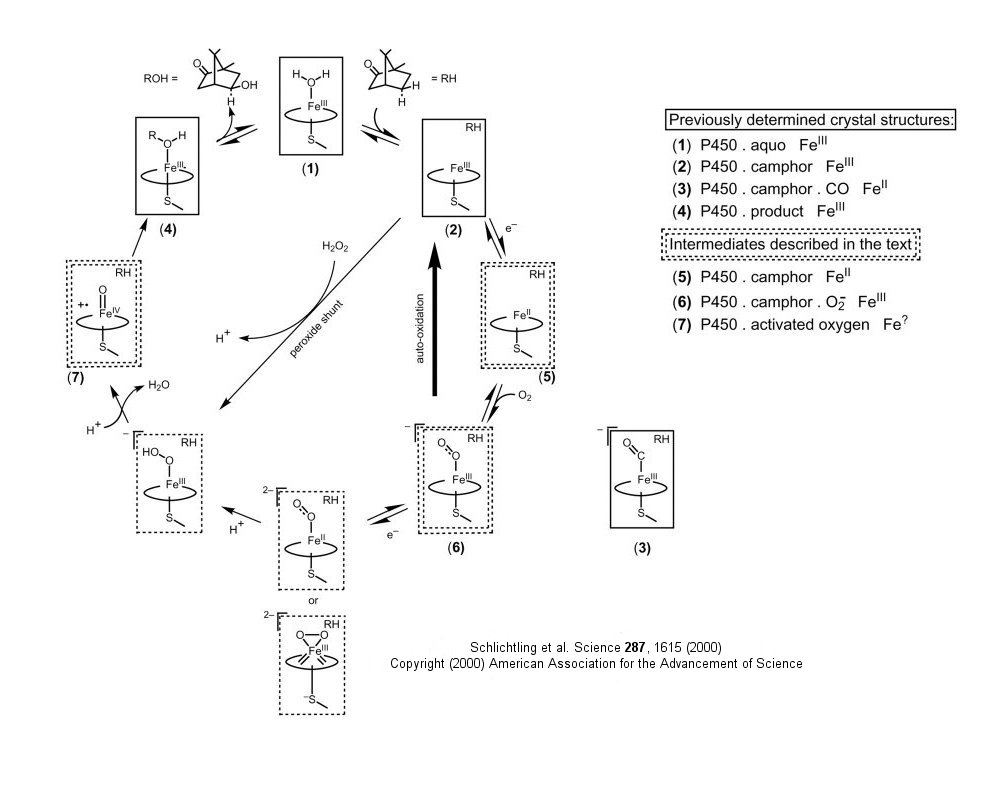
Chime Molecular Modeling: Cytochrome P450cam
DIOXYGENASES
An example of a dioxygenase is the cyclooxygenase activity of prostaglandin synthase. This enzyme, often
just called cycloxygenase or COX, is an integral membrane protein found in the ER membrane, and is a homodimer (with two hemes).
It catalyzes two different reactions. One is the addition of two dioxygens to arachidonic acid - 20:4D5, 8, 11, 15 (which is liberated from the C2 position of
phospholipid membranes by phospholipase A2 upon appropriate signaling) to form prostaglandin PGG2. This molecule,
with 5 chiral centers, arises from arachidonic acid, which has one. The cyclooxygenase activity is buried in the membrane,
from where the arachidonic acid can readily access its active site. The active site is at the end of a hydrophobic channel
(arachidonic acid binding site) and stretches from the membrane-binding region to a buried heme. PGG2 can
be further metabolized to PGH2 by the addition of two electrons to PGG2 by the hydroperoxidase activity
of the enzyme, located at the other end of the enzyme. This activity forms an alcohol from the peroxide functional group
in PGG2. There is one heme per momomer, which acts in both the cyclooxygenase and peroxidase activities.
Each monomer of the dimer has both enzymatic activities.
The reaction mechanism appears to involve a free radical. A possible free radical mechanism, based
on recent work by Marnett et al. and Kiefer et al. is shown and summarized below.
- The carboxylate of arachidonic acid is coordinated to Arg 120 and Tyr 355.
- The C13 pro(S) H atom of arachidonic acid is close to Tyr 385 which allows its abstraction.
- This results in a radical centered on C11 that reacts with dioxygen to form a peroxyl radical
- Attack by dioxgen at C11 occurs from the side of the substrate opposite to that of hydrogen abstraction
- The oxygen radical at C11 cyclizes by attacking C9.
The C13 proS hydrogen atom (not proton) is remove from bound arachidonic acid by a free radical form of
Tyr 385, which acts as an oxidizing agent. A site-specific mutants in which Tyr 385 is replaced by Phe is inacive.
How is the Tyr free radical formed? Based on single electron standard reduction potential (0.9 V for Tyr and -0.2 to
+ 0.2 V for Fe3+ in the bound heme, it appears that the heme iron is not a potent enough oxidizing agent to accomplish
this task. However, oxygen bound to the heme iron could be converted to a peroxide and form an Fe4+-oxo complex
much like we saw step 7 of a possible reaction pathways for cytoP450cam. The Fe4+ ion is a more potent oxidzing agent (standard reduction potential of approximately 1V, sufficient
for oxidation of Tyr 385. Another possibility is that the peroxide activator (in the formation of the ferryl-oxo
ligand) is NO (nitric oxide, a free radical). NO is formed by immune cells (like macrophages) on immune activation.
The NO might react with superoxide (also a radical, possibly formed during an oxidative burst in macrophages during immune
stimulation) to form peroxynitrite (NO3-). This can donate an oxo group to the Fe3+
to form the Fe4+-oxo complex, which could then oxidize Tyr to the free radical form. There might be other
mechanisms as well to generate the Tyr free radical, since just adding organic peroxides to the enzyme will generate it..
After abstraction of the proS H atoms, a carbon-centered free radical at C11 results which reacts with oxygen as shown below.
The exact form of oxygen that reacts is unclear, but presumably is either a peroxy or activated singlet form.
Figure: Prostatglandin Synthesis: Possible Mechanism
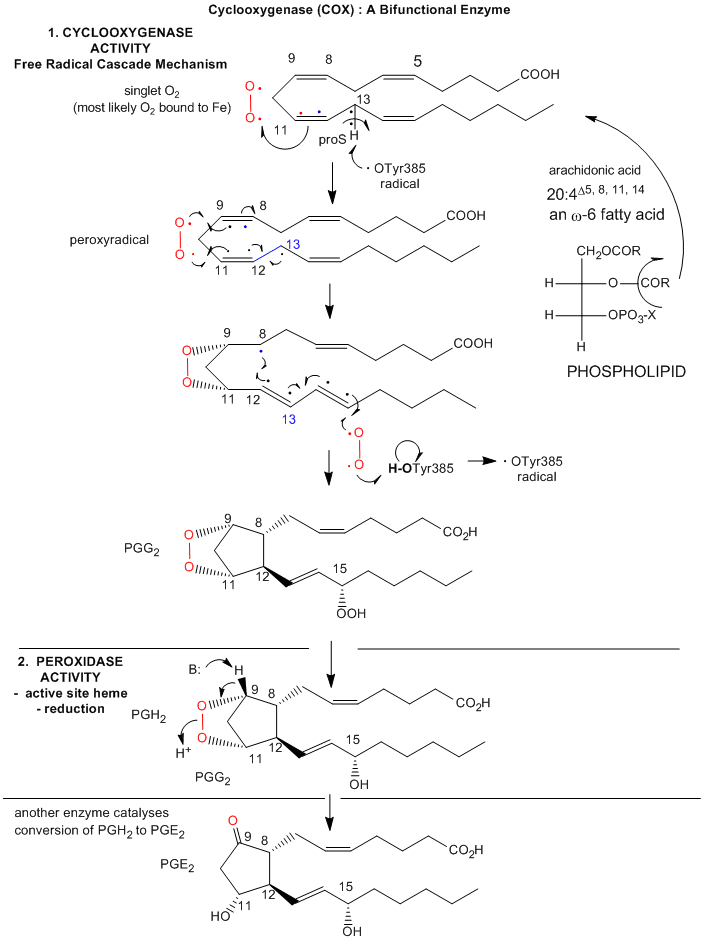
Prostaglandins, which were first isolated from prostate glands, serve as powerful, but labile local hormones
which are mediators of pain, inflammation, immune and clotting activity. The cyclooxygenase activity is
inhibited by aspirin, which probably accounts for most of its anti-inflammatory and analgesic properties. Aspirin, acetylsalicylic
acid, acetylates a reactive Ser 530 in the active site. Another nonsteroidal anti-inflammatory drug (NSAID) with similar
properties is Ibuprofen (Advil). Acetaminophen (Tylenol) is also considered a member of this drug class, even
though it doesn't have anti-inflamatory properties. The question has arisen as to why. It now turns out that there
are apparently three different types of COX, I, II, and III. COX III is expressed in the brain, and might be involved
in pain pathways. Acetaminophen appears to work on this COX, as shown in the chart below (Bazan et al.).
Cyclooxygenase Activities
COX |
Expression |
Function |
Inhibitors |
COX 1 |
constitutively |
organ pain, platelet function, stomach protection |
NSAIDs including aspirion |
COX 2 |
induced by growth factors, neurotransmitters, inflammatory cytokines, oxidative stress, injury. Constitutively
in brain, kidney |
Inducible COX2: inflammation, pain, fever Constitutive COX2: synaptic plasticity |
NSAIDs, COX 2 inhibitors including celecoxib (Celobrex ) which has few GI problems associated with its use |
COX 3 |
constitutively, high in brain, heart |
pain pathways, not inflammation pathways |
acetaminophen (no GI problems, great fever reducer), some NSAIDs |
Chime Molecular Modeling: Cyclooxygenase I and Nonsteroidal anti-inflammatory drugs (NSAID)
Fish n-3 fatty acids and health
We mentioned the importance of arachidonic acid in signal transduction in the lipid chapter. In addition, the importance
of n-3 fatty acids to health was discussed as well. As mentioned above, arachidonic acid is cleaved from the C2 or sn-2
position of membrane phospholipids and modified by cyclooxygenase or lipoxygenase to form prostaglandins and leukotrienes,
both potent local biological mediators. Linoleic acid and 22:6n-3 (DHA) are also found in membrane phospholipids at
the sn-2 position. What is the mechanism for the health-protective effects of n-3 fatty acids like DHA?
In human tissue, DHA, 22:6n-3 or 22:6D5,8,11,14,17,20 is the most abundant n-3 PUFA.
Since it is synthesized from linolenic acid (as is EPA), a deficiency of linolenic acid in the diet will lead to lowered levels
of 22:6n-3 in tissues, with ensuing health effects. Since these lipids are involved in membrane structure, signal transduction,
and hormone synthesis, diverse effects of dietary n-3 PUFA deficiency will be observed. 50% of all fatty acids in the
sn1 and sn2 position of membrane phospholipids of rod outer segments (in the retina) are 22:6(n-3). Cognitive dysfunctions
(loss of memory, etc.) have been linked to decreased levels of 22:6(n-3) in the brain. This fatty acid binds to retinoid
X receptors which then activate (through linked binding reactions) nuclear receptors, leading to alterations in gene transcription
in the CNS.
In other tissues, 22:6(n-3) rarely exceeds 10% of membrane fatty acids, but this percentage can be increased in cells with
increases in a precursor, 20:5(n-3). DHA might affect lipid rafts in the membrane, which would affect movement
of important membrane protein receptors (and associated proteins) in the membrane, altering cell response to environmental
stimuli. DHA and EPA affect arachadonic acid conversion to prostaglandins and leukotrienes. EPA binds less tightly
to cycloxygenase I and is a poor substrate for the enzyme, both effects which inhibit the formation of prostaglandins and
signaling processes mediated by them. This explains why n-3 fatty acids have anti-inflamatory effects.
In addition, n-3 fatty acids have noticeable effects on gene transcription, which remain as long as these fatty acids are
present in high levels in the diet These and other fatty acids bind to fatty acid-activated transcription factors called
PPARs (peroxisome proliferator receptors - alpha, beta and gamma 1 and 2). These receptors regulate, through alterations
in gene expression, proteins involved in lipid metabolism. Other fatty acid-dependent transcription factors are known
as well. PPAR's bind 20:5(n-3) with a micromolar Kd and change the conformation of the protein to a form than can bind
other proteins, ultimately altering gene expression.
Table: Biological Effects of n-3 polyunsaturated fatty acids EPA (20:5) and DHA (22:6)
Organ(s) |
Effect |
Mechanism acts through |
central nervous system |
improve cognitive function |
membrane composition; retinoic X receptor alpha |
retina |
improve acuity |
membrane composition |
immune |
immunosuppressive; antinflamatory |
membrane composition; rafts |
cardiovascular |
anti-arrhythmia; anti-clotting |
membrane composition; rafts; eicosanoids |
serum lipids |
lowers triglycerides (risk factor for cardiovas. dis) |
peroxisome proliferator receptor alpha and gamma |
liver |
decrease lipid synthesis; increase fatty acid oxid. decrease VLDL synthesis |
sterol reg. element bind. protein; PPAR alpha PPAR alpha |
Adapted from Jump D. The Biochemistry of n-3 Polyunsaturated fatty acids. J. Biol. Chem. 277, pg 8755 (200)
OXIDASES.
This class of enzymes does not incorporate dioxygen into an organic substrate. Rather it accepts electrons
released from an organic substrate, through intemediate electron carriers (such as ubiquinone and cytochrome C) to form superoxide
(as in NADPH-oxidase), hydrogen peroxide (as in xanthine oxidase) or water (as in cytochrome C oxidase). The mechanism of
cytochrome C oxidase again supports our expectations about enzymes that use dioxygen. Dioxygen binds metals in the enzyme.
One oxygen atom binds a heme Fe2+ of cytochrome a3 which is bound to the enzyme, while the other binds
a Cu1+ of Cu B. All oxygyen reduction intermediates remain bound to the enzyme. Four electrons
are added from four different cytochrome C molecules, which serve as mobile carriers of electrons.
Chime Molecule Modeling: Cytochrome C Oxidase
Figure: Oxidases: Examples
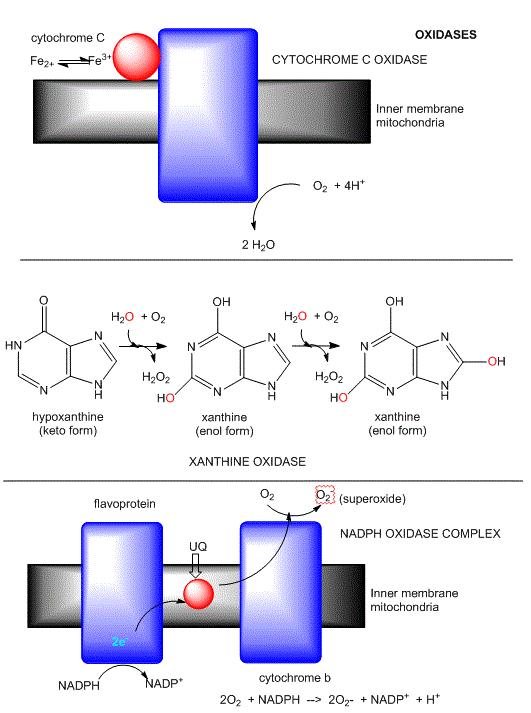
THE AMAZING HEME PROTEINS:
So far in this course, we have examined three different kinds of heme proteins..
- The first, hemoglobin (and myglobin) serve as carriers of dioxygen. Even though they bind one of the
best oxidizing agents around (dioxygen), the heme Fe2+ does not get oxidized to Fe3+. If it does, as
in the case of met-Hb, the protein looses it ability to carry oxygen.
Figure: hemoglobin (and myglobin) serve as carriers of dioxygen
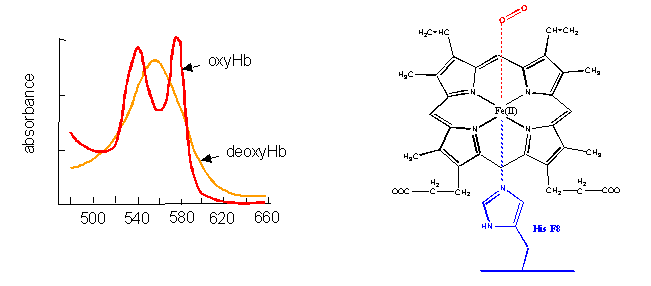
- Cytochrome C, on the other hand, does not bind dioxygen but rather serves as a carrier of electrons which
get passed to dioxygen in Cytochrome C oxidase. Its Fe ion readily cycles between the 2+ and 3+ states as it serves as an
electron carrier.
Figure: Cytochrome C
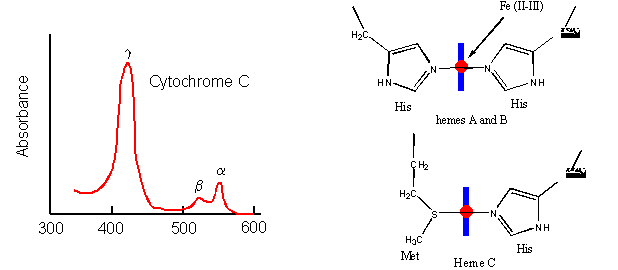
- Finally, the Fe2+ in the heme of the cytochrome P450s (so named since they have an absorbance
maximum at 450 nm when they bind CO) does both. It binds dioxygen and cycles between the 2+ and 3+ states as it activated
dixoxygen for hydroxylation reactions.
Figure: cytochrome P450s
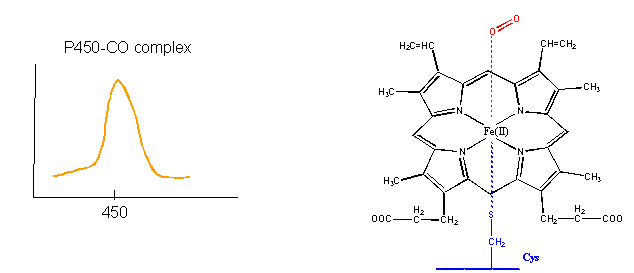
How could heme serve such diverse functions? We can explain this by referring to one of the main themes
of the course - structure mediates function. The environment of each heme must be different.
Clearly the protein ligands coordinating the Fe ions are different. The 5th ligand is the proximal His in hemoglobin while
dioxygen binds to the 6th site. In cytochrome C, the 5th and 6th ligands are His and Met, respectively. In cytochrome
P450, the 5th site is occupied by Cys, and the 6th by dioxygen. Presumably the environments surrounding the hemes are different
as well. Once again, we have seen analogous example in which chemical properties are influenced by the microenvironment. The
pKa of a given amino acid side chain can vary considerably depending on the polarity of the local environment. Likewise, the
standard reduction potential of tightly bound FAD/FADH2 depends on the microenvironment.
TRANSITION METALS AND CELLS
As we have seen (from the study of heme proteins and the oxidative enzymes of cells), transition metals
such as Fe, Zn, and Cu have vital biological roles as binding sites and cofactors in many reactions. Yet they also pose
problems since they can lead to oxidative damage in cells. As we saw with cytoplasmic metallothioniens, which bind to
heavy metals and protect the cell from such damage, many proteins are involved in binding and regulation of transition metals
in the cell. Integral membrane proteins are required to bind and transport these cations into the cytoplasm. Other proteins
act as sensors of transition ion concentration (such as latent transcription factors which bind heavy metals and become active
transcription factors for metallothioniens. Others act as chaperone proteins which bind metal ions and transfers
them to apometalloproteins. Recent work has suggested transporters and chaperones involved in metal ion biology bind
these ions with unusual coordination geometry, which presumably facilitates transfer of the ion to the apo-target protein.
The transition metals Zn and Fe are often found in E. Coli at a concentration of 0.1 mM, compared to Cu
and Mn which are present at concentrations from 10 to 100 mM. Also,
about one third of all proteins demonstrate specific binding of metal ions and can be classified as metalloproteins.
Mass balance suggests that metal ions would be distributed in proteins with low, intermediate, and high metal binding affinity
as well as in free pools, which which potentially be toxic to cells. Metalloproteins, depending on their Kd for metal
ion binding, would hence be in various state of ligation. The free concentration of some ions (Cu and Zn) is so low
that newly synthesized apoproteins which bind these ions would not obtain the ion from the free pool. In such cases,
metal chaperones would be required.