We can apply what we learned about catalysis by small molecules to enzyme-catalyzed reactions. To understand
the mechanism of an enzyme-catalyzed reaction, we try to alter as many variables, one at a time, and ascertain the effects
of the changes on the activity of the enzyme. Kinetic methods can be used to obtain data from which inferences about the mechanism
can be made. Obviously, crystal structures of the enzyme in the presence and absence of a competitive inhibitor give abundant
information about possible mechanisms. It is amazing, however, how much information about enzyme mechanism can be gained
even if all you have is a blender, a stopwatch, an impure enzyme, and a few substrates and inhibiting reagents. Systematically,
the kineticist and organic chemist can change:
- the substrate - for example, changing the leaving group or acyl sustituents of a hydrolyzable
substrate;
- the pH or ionic strength - which can give data about general acids/bases in the active
site;
- the enzyme - by chemical modification of specific amino acids, or through site-specific
mutagenesis;
- the solvent - as will be discussed in the next chapter section .
We will explore in detail the mechanisms of three enzymes. For carboxypeptidase, we will study possible
mechanisms for the cleavage of C-terminal hydrophobic amino acids from a peptide. For lysozyme, we will study the structural
features of the enzyme and substrates along with the mechanism for cleavage of glycosidic links in bacterial peptidoglycan
cell walls. For chymotrypsin, we will study experiments which vary the substrate, pH, and the enzyme and infer from
this information about a mechanism consistent with the experimental data.
CARBOXYPEPTIDASE
A peptide substrate binds at the active site of the enzyme. X-ray structures of the enzyme with and without
a competitive inhibitor show a large conformational change at the active site when inhibitor or substrate is bound. Without
inhibitor, several waters occupy the active site. When inhibitor and presumably substrate are bound, the water leaves (which
is entropically favored), and Tyr 248 swings around from near the surface of the protein in the absence of a molecule in the
active site to interact with the carboxyl group of the bound molecule, a distance of motion equal to about 1/4 the diameter
of the protein. This effectively closes off the active site and expels the water. A Zn2+ ion is present at the
active site. It is bound by His 69, His 196, Glu 72, and finally a water molecule as the fourth ligand. A hydrophobic pocket
which interacts with the phenolic group of the substrate accounts for the specificity of the protein.
In the catalytic mechanism, Zn2+ helps polarize the labile amide bond, while Glu 270, acting
as a general base, which along with Zn2+ helps promote dissociation of a proton from the bound water, making it
a better nucleophile. Water attacks the elctrophilic carbon of the sessile bond, with Glu 270 acting as a general base
catalyst. The tetrahedral intermediate then collapses, expelling the leaving amine group, which picks up a proton from Glu
270, which now acts as a general acid catalyst. People used to believe that Tyr 248 acted as a general acid, but mutagenesis
showed that Tyr 248 can be replaced with Phe 248 without significant effect on the rate of the reaction.
LYSOZYME
This enzyme, found in cells and
secretions of vertebrates but also in viruses which infect bacteria, cleaves peptidoglycan GlcNAc (b
1->4) MurNAc repeat linkages (NAG-NAM) in the cell walls of bacteria and the GlcNAc (b 1->4) GlcNAc (poly-NAG) in chitin, found in the cells walls of certain fungi. Since these polymers
are hydrophilic, the active site of the enzyme would be expected to contain a solvent-accessible channel into which the polymer
could bind. The crystal structures of lysozyme and complexes of lysozyme and NAG have been solved to high resolution.
The inhibitors and substrates form strong H bonds and some hydrophobic interactions with the enzyme cleft. Kinetic
studies using (NAG)n polymers show a sharp increase in kcat as n increases from 4 to 5.
The kcat for NAG6 and (NAG-NAM)3 are similar. Models studies have shown that
for catalysis to occur, (NAG-NAM)3 binds to the active site with each sugar in the chair conformation except the
fourth which is distorted to a half chair form, which labilizes the glycosidic link between the 4th and 5th sugars.
Additional studies show that if the sugars the fit into the binding site are labeled A-F, then because of the bulky lactyl
substituent on the NAM, residues C and E can not be NAM, which suggests that B, D and F must be NAM residues. Cleavage
occurs between residues D and E.
A review of the chemistry of glycosidic bond (an acetal) formation and cleavage shows the acetal
cleavage is catalyzed by acids and proceeds by way of an oxonium ion which exists in resonance form as a carbocation.
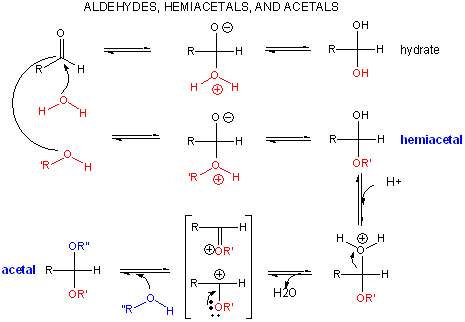
Catalysis by the enzyme involves Glu 35 and Asp 52 which are in the active site. Asp
52 is surrounded by polar groups but Glu 35 is in a hydrophobic environment. This should increase the apparent
pKa of Glu 35, making it less likely to donate a proton and acquire a negative charge at low pH values, making it a better
general acid at higher pH values. The general mechanism appears to involve:
-
binding of a hexasaccharide unit of the peptidoglycan with concomitant distortion of the D
NAM.
-
protonation of the sessile acetal O by the general acid Glu 35 (with the elevated pKa), which
facilitates cleavage of the glycosidic link and formation of the resonant stabilized oxonium ion.
-
Asp 52 stabilizes the positive oxonium through electrostatic catalysis. The
distorted half-chair form of the D NAM stabilizes the oxonium which requires co-planarity of the substituents attached to
the sp2 hybridized carbon of the carbocation resonant form (much like we saw with the planar peptide bond).
-
water attacks the stablized carbocation, forming the hemiacetal with release of the extra proton
from water to the deprotonated Glu 35 reforming the general acid catalysis.
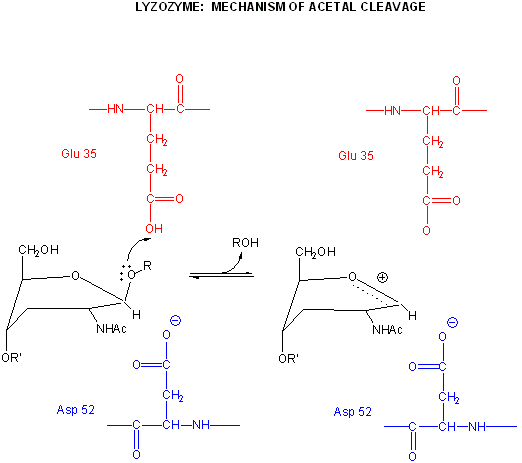
Binding and distortion of the D substituent of the substrate (to the half chair form as shown
above) occurs before catalysis. Since this distortion helps stabilize the oxonium ion intermediate, it presumably stabilizes
the transition state as well. Hence this enzyme appears to bind the transition state more tightly than the free, undistorted
substrate, which is yet another method of catalysis.
pH studies show that side chains with pKa's of 3.5 and 6.3 are required for activity.
These presumably correspond to Asp 52 and Glu 35, respectively. If the carboxy groups of lysozyme are chemically modified
in the presence of a competitive inhibitor of the enzyme, the only protected carboxy groups are Asp 52 and Glu 35.
A recent paper suggests an alternative mechanism to the Phillips mechanism above. In
this case, Asp 52 acts as a nucleophilic catalysis and forms a covalent bond with NAM, expelling a NAG leaving group with
Glu 35 acting as a general acid. This alternative mechanism also is consistent with other b-glycosidic bond cleavage enzyme. Substrate distortion is also important in this alternative mechanism.
Figure: alternative mechanism
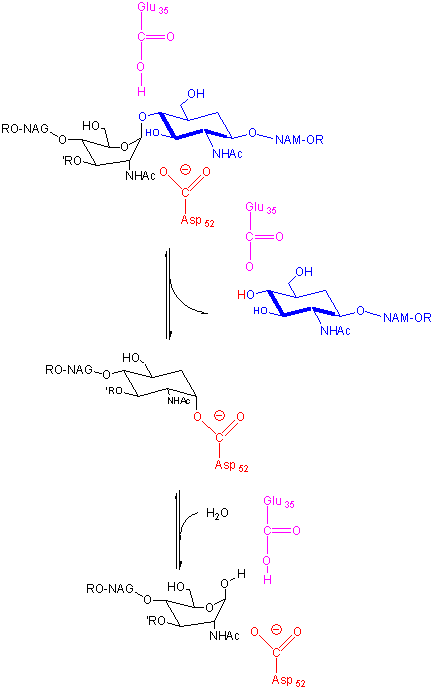
CHYMOTRYPSIN
Chymotrypsin, a protease, cleaves amides as well as small ester substrates after aromatic residues. The
following data using different chymotrypsin substrates suggests a covalent intermediate occurs on chymotrypsin catalyzed cleavage
of esters and amides.
- changing the substrate - for example changing the leaving group or acyl sustituents
of a hydrolyzable substrate:
Chymotrypsin substrate cleavage, 25oC, pH 7.9 |
kinetic constants |
Acetyl-Tyr-Gly-amide |
Acetyl-Tyr-O Ethylester |
Ester/Amide |
kcat (s-1) |
0.50 |
193 |
390 |
Km (M) |
0.023 |
0.0007 |
0.03 |
kcat/Km (M-1s-1) |
22 |
280,000 |
12,700 |
Kinetic constants for chymotrypsin cleavage of N-acetyl-L-Trp Derivatives - N-acetyl-L-Trp-X |
X |
kcat (s-1) |
Km x 103 (M) |
-OCH2CH3 |
27 |
0.097 |
-OCH3 |
28 |
0.095 |
-p-nitrophenol |
31 |
0.002 |
-NH2 |
0.026 |
7.3 |
- the kcat and kcat/Km are larger and the Km smaller for ester
substrates compared to amide substrates, suggesting that amides are more difficult to hydrolyze (tables 1 and 2 above). This
is expected given the poorer leaving group of the amide.
- the kcat for the hydrolysis of ester substrates doesn't depend on the nature of the leaving
group (i.e. whether it is a poorer leaving group such as methoxy or a better leaving group such as p-nitrophenolate) suggesting
that this step is not the rate limiting step for ester cleavage. (Table 2). Without the enzyme, p-nitrophenyl esters
are cleaved much more rapidly than methyl esters. Therefore deacylation must be rate limiting. But deacylation of what? If
water was the nucleophile, release of the leaving group would result in both products, the free carboxyl group and the amine
being formed simultaneously. This suggests an acyl-enzyme covalent intermediate.
- When the acyl end of the ester substrate is changed, without changing the leaving group (a p-nitrophenyl
group), a covalent intermediate can be trapped. Specifically, the deacylation of a trimethyacetyl group is much slower than
an acetyl group. It is so slow that a 14C-labeled trimethylacetyl-labeled chymotrypsin intermediate can be
isolated after incubation of chymotrypsin with 14C-labeled p-nitrophenyltrimethylacetate using gel filtration chromatography.
We have seen a kinetic mechanism consistent with these ideas before. The reaction equations are
shown below:
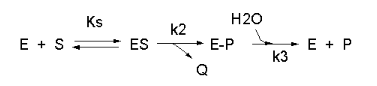
In this reaction, a substrate S might interact with E to form a complex, which then is cleaved to products
P and Q. Q is released from the enzyme, but P might stay covalently attached, until it is expelled. This conforms exactly
to the mechanism described above. For chymotrypsin-catalyzed cleavage, the step characterized by k2
is the acylation step (with release of the leaving group such as p-nitrophenol in Lab 5). The step characterized by
k3 is the deacylation step in which water attacks the acyl enzyme to release product P (free phosphate in
Lab 5). In class and for homework you derived the following equation::
Equation 7: v = [(k2k3)/(k2 + k3)]EoS/[Ks(k3)/(k2+k3)]
+ S
As mentioned above, for hydrolysis of ester substrates, which have better leaving groups compared to amides,
deacylation is rate limiting, ( k3<<k2). Then equation 7 becomes
v = k3EoS/[Ks(k3)/(k2) + S]
Vm = k3Eo and Km = Ks(k3)/(k2)
For amide hydrolysis, as mentioned above, acylation can be rate-limiting (k2<<k3).
Then equation 7 becomes:
v = k2EoS/[Ks+ S]
Vm = k2Eo and Km = Ks
Just as we saw before for the rapid equilibrium assumption (when ES falls apart to E + S more
quickly than it goes to product), Km = Ks in amide hydrolysis.
- changing the pH or ionic strength - which can give data about general acids/bases
in the active site:
- a graph of kcat as a function of pH indicates that a group of pKa of approx. 6 must be deprotonated
to express activity (i.e. Vm/2 is at about pH 6). This suggests that an active site histidine is necessary, which if
it must be deprotonated to express activity, must be acting as a general base.
- a graph of kcat/Km shows a bell-shaped curve indicating the necessity of a deprotonated
side chain with a pKa of about 6 (i.e. the same His above) and a group which must be protonated with a pKa of about 10. This
turns out to be an N terminal Ile (actually at the 16 position in the inactive precursor of chymotrypsin called chymotrypsinogen,
which on activation of chymotrypsinogen loses the first 15 amino acids by selective proteolysis), which must be protonated
to form a stabilizing salt bridge in the protein.
pH effects on trypsin and chymotrypsin: a mathematical derivation Note: A new theoretical computer program, called THEMATICS (theoretical microscopic titration curves) has been developed to calculate the titration curves for all ionizable groups
in a protein. When performed on test proteins, those amino acids that showed anomalous curves (flattened compared to
normal titration curves) where usually found in the active site of the protein. The flattened curves show that the amino
acid is partially protonated over a wider range of pH then theoretically expected. The program can be used to predict
active site regions on protein of known structure but unknown function, and will be useful in the emerging field of proteomics.
(figure below from Proc. Natl. Acad. Sci. USA, Vol. 98, Issue 22, 12473-12478, October 23, 2001)
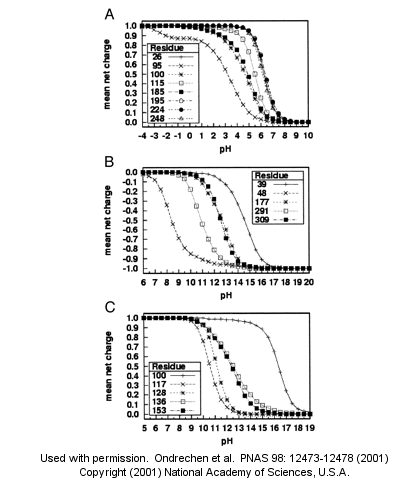
Fig. 1. Sample theoretical titration curves. Predicted mean net charge as a function of pH. (A) All of
the histidine residues in the A chain of TIM: His-26 (+), His-95 (×), His-100 (*), His-115 (open square), His-185 (filled
square), His-195 (open circle), His-224 (filled circle), and His-248 (). (B) Selected tyrosine residues of AR: Tyr-39 (+),
Tyr-48 (×), Tyr-177 (*), Tyr-291 (open square), and Tyr-309 (filled square). (C) Selected lysine residues of PMI: Lys-100
(+), Lys-117 (×), Lys-128 (*), Lys-136 (open square), and Lys-153 (filled square). TIM, triosephosphate isomerase; AR,
aldose reductase; PMI, phosphomannose isomerase;
- the enzyme - by chemical modification of specific amino acids, or through site-specific
mutagenesis:
- modification of chyrmotrypsin (and many other proteases) with diisopropylphosphofluoridate (DIPF) modifies
one of many Ser residues (Ser 195), suggesting that it is hypernucleophilic. and probably the amino acid which attacks the
carbonyl C in the substrate, forming the acyl-intermediate.
Figure: diisopropylphosphofluoridate
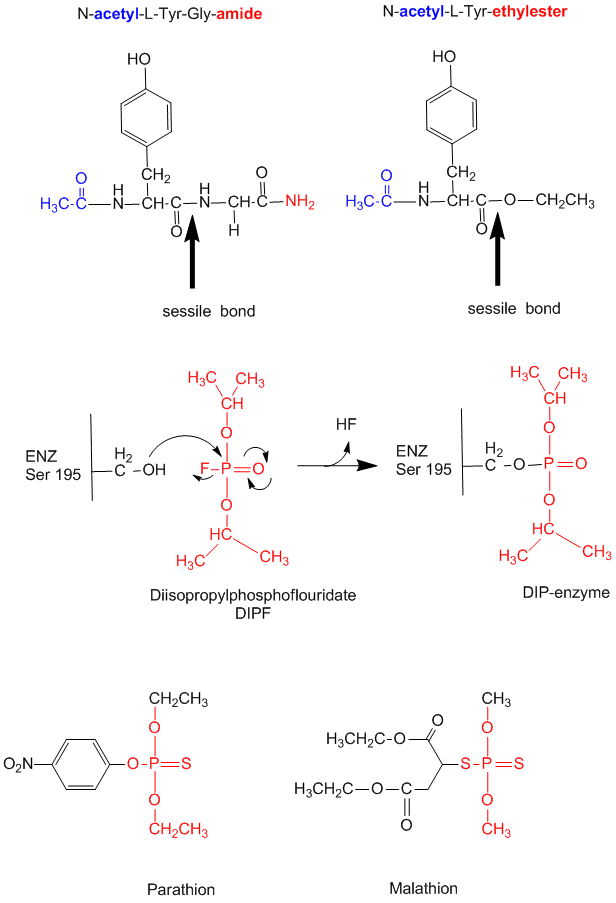
Malathion and ethyl parathion (organic phosphate pesticides) have similar structures and reactivities as DIPF, and selectively inhibit serine proteases
in insects.
- modification of the enzyme with tos-L-Phe-chloromethyl ketone inactives the enzyme with a 1:1 stoichiometry
which results in a modified His.
Figure: tos-L-Phe-chloromethyl ketone
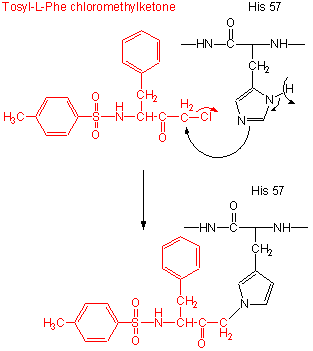
- comparison of the primary sequence of many proteases show that three residues are invariant: Ser 195,
His 57, Asp 102.
- site-specific mutagenesis show that if Ser 195 is changed to Ala 195, the enzymatic activity is almost
reduced to background.
Figure: Serine Protease Mechanism
- The deprotonated His 57 acts as a general base to abstract a proton from Ser 195, enhancing its nucleophilicty
as it attacks the electrophilic C of the amide or ester link, creating the oxyanion tetrahedral intermediate. Asp 102 acts
electrostatically to stabilize the positive charge on the His.
- The oxyanion collapses back to form a double bond between the O and the original carbonyl C, with the
amine product as the leaving group. The protonated His 57 acts as a general acid donating a proton to the amine leaving group,
regenerating the unprotonated His 57.
- The mechanism repeats itself only now with water as the nucleophile, which attacks the acyl-enzyme intermediate,
to form the tetrahedral intermediate.
- The intermediate collapses again, releasing the E-SerO- as the leaving group which gets reprotonated
by His 57, regenerating both His 57 and Ser 195 in the normal protonation state. The enzyme is now ready for another catalytic
round of activity.
In short, all the catalytic mechanisms we encountered previously are at play in chymotrypsin catalysis.
These include nucleophilic catalysis (with the Ser 195 forming a covalent intermediate with the substrates), general acid/base
catalysis with His 57, and loosely, electrostatic catalysis with Asp 102 stabilizing not the transition state or intermediate,
but the protonated form of Hs 57. One final mechanism is at work. The enzyme does indeed bind the transition state more tightly
than the substrate. Crystal structures with poor "pseudo"-substrates that get trapped as partial tetrahedrally-distorted substrates
of the enzyme and with inhibitors show that the oxyanion intermediate, and hence presumably the TS, can form H-bonds with
the amide H (from the main chain) of Gly 193 and Ser 195. These can not be made to the trigonal, sp2 hybridized substrate.
In the enzyme alone, the hole into which the oxyanion intermediate and TS would be placed is not occupied. This oxyanion hole
is occupied in the tetrahedral intermediate.
Figure: oxyanion hole in serine proteases: TS stabilzation
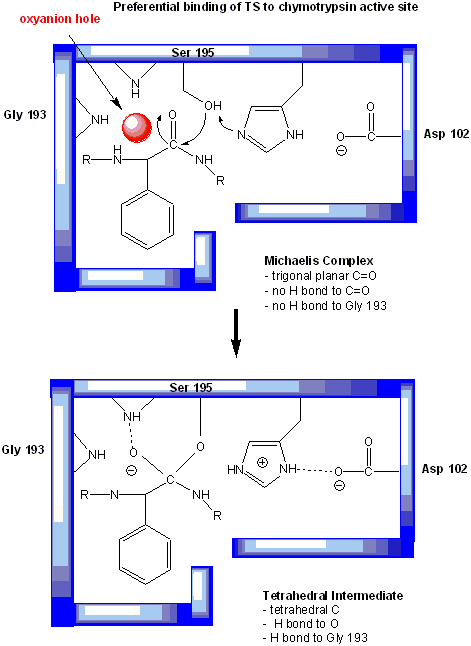
Web Links:
Many enzymes have active site serines which act as nucleophilic catalysts in nucleophilic substitution reactions
(usually hydrolysis). One such enzyme is acetylcholine esterase which cleaves the neurotransmitter acetylcholine in
the synapse of the neuromuscular junction. The transmitter leads to muscle contraction when it binds its receptor on
the muscle cell surface. The transmitter must not reside too long in the synapse, otherwise muscle contraction will
continue in an uncontrolled fashion. To prevent this, a hydrolytic enzyme, acetylcholine esterase, a serine esterase
found in the synapse, cleaves the transmitter, at rates close to diffusion controlled. Diisopropylphosphofluoridate
(DIPF) also inhibits this enzyme which effectively makes it a potent chemical warfare agent. An even more fluoride-based
inhibitor of this enzyme, sarin, is the most potent lethal chemical agent of this class known. Only 1 mg is necessary
to kill a human being.
Figure: sarin
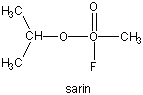
Proteases Mechanisms
Serine proteases are just one type of endoproteases. However, they are extremely abundant in both prokaryotes
and eukaryotes. Protease A, a chymotrypin-like protease from Stremptomyces griseus, has a very different primary
sequence than chymotrypsin, but its overall tertiary structure is quite similar to chymotrypsin, The positions of the
catalytic triad amino acids in the primary sequences of the protein are very similar, indicating that the genes for the proteins
diverged from a common precursor gene. In contrast, subtilisin, a serine protease from B. Subtilis, has both
limited sequence and tertiary structure homology to chymotrypsin. However, when folded it also has a catalytic triad
(Ser 221 - His 64 - Asp 32) similar to that of chymotrypsin (Ser 195 - His 57 - Asp 102).
Proteases have multiple functions, other than in digestion, including degrading old or misfolded proteins
and activating precursor proteins (such as clotting proteases and proteases involved in programmed cell death). In general,
four different classes of proteases have been found, based on residues found in their active sites. Proteases can also
be integral membrane proteins, and carry out their activities in the hydrophobic environment of the membrane. For example,
aberrant cleavage of the amyloid precursor protein by the membrane protease presenillin can lead to the development of Alzheimers.
Figure: amyloid precursor protein
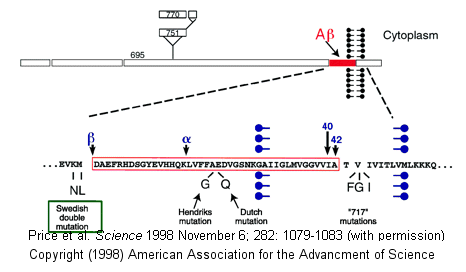
Classification of Proteases
Class (active site) |
Location |
Examples |
Serine/Threonine |
soluble |
trypsin, chymotrypsin, subtilisin, elastase, clotting enzymes, proteasome |
membrane |
Rhomboid family |
Aspartic |
soluble |
pepsin, cathepsin, renin, HIV protease |
membrane |
b-secretase (BACE), presenilin I, signal peptide peptidase |
Cysteinyl |
soluble |
bromelain, papain, cathespsins, caspases |
membrane |
? |
Metallo |
soluble |
thermolysin, angiotensin converting enzyme |
membrane |
S2P family |
b-secretase (BACE) is a membrane protein that contains two necessary Asp residues in
its ectodomain (extracellular domain) which are used in the first cleavage of the N terminal domain of the beta amyloid
precursor protein to release a soluble, N-terminal fragment of about 100,000 MW. g-secretase,
necessary for the second cleavage which frees the Ab peptide is a heterotetramer composed of presenillin-1,
nicastrin, APH-1 and PEN-2, and is located in neural plasma membranes and endoplasmic reticulum. The Ab
peptide moves to the extracellular side of the neural membrane where it aggregates. The remaining cytoplasmic part of
the beta-amyloid precursor protein may regulate transcription. The presenillin subunit has protease activity.
g-secretase also cleaves another cell surface receptor protein, Notch. When this receptor has
bound an extracelluar ligand, g-secretase cleaves Notch within the cytoplasm, and the released fragment
modifies gene transcription. The APH-1 subunit appears to inhibit presenilin protease activity while PEN-2 promotes
it. Inhibiting g-secretase would be an effect treatment for Alzheimers, but might have serious
side effects since Notch processing would also be affected.
Figure: Cleavage of beta amyloid precursor protein: protease and cofactors
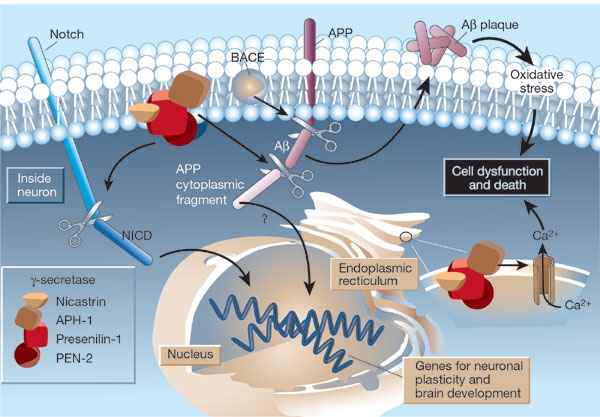
The g-secretase protein
quartet, and its roles in brain development and Alzheimer's disease. Presenilin-1, nicastrin, APH-1 and PEN-2 form a functional
g-secretase complex, located in the plasma membrane and endoplasmic
reticulum (ER) of neurons. The complex cleaves Notch (left) to generate a fragment (NICD) that moves to the nucleus and regulates
the expression of genes involved in brain development and adult neuronal plasticity. The complex also helps in generating
the amyloid b-peptide (Ab; centre). This involves an initial cleavage of the amyloid precursor protein (APP) by an enzyme called
BACE (or b-secretase). The g-secretase then liberates Ab, as well
as an APP cytoplasmic fragment, which may move to the nucleus and regulate gene expression. Mutations in presenilin-1 that
cause early-onset Alzheimer's disease enhance g-secretase
activity and Ab production, and also perturb the ER calcium
balance. Consequent neuronal degeneration may result from membrane-associated oxidative stress, induced by aggregating forms
of Ab (which create Ab plaques), and by the perturbed calcium balance. Reprinted by permission from Nature: Mattson, M. Nature. 422, 385-387 (2003) |
How do integral membrane protease catalyze the hydrolysis (using water) of transmembrane domains in proteins,
given the hydrophobic environment of the bilayer? The rhomboid class of membrane proteases, which are found in
prokaryotic and eukaryotic cells, is one of the most conserved membrane proteins in nature. Sequence comparison
of rhomboid proteins shows a possible catalytic serine residue that is buried to the same depth in the bilayer as the cleavage
site for rhomboid protein substrates. In addition the proteases share conserved amino acids similar to the catalytic
triad of soluble serine proteases, with the critical amino acid localized on different membrane-spanning regions of the protein.
The membrane triad amino acids are Ser, His and Asn (instead of Asp as in classical serine proteases). Classical serine
protease inhibitor prevent cleavage of membrane protein substrates, but given the difficulty of reconstituting a functional
purified rhomboid protein in vitro, the identity of specific amino acids in the catalytic mechanism is inferential
at present.
Helical wheel diagrams of putative transmembrane domains of the rhomboids (determined through hydropathy plots)
show several to be amphiphilic. This might allow water, necessary for hydrolysis, to enter into the catalytic active
site of the enzyme, although as was noted before, water has a reasonably high permeability through a membrane bilayer.
The chief requirement for protein substrates of rhomboids is the presence of a transmembrane domain in the target protein.
No specific amino acid sequence seems to be required for specificity of one particular substrate, the drosophila transmembrane
protein spitz found in Golgi membranes. On cleavage of this protein, the remaining part of the protein is released as
a water soluble protein to the lumen of the Golgi where it can eventually be released from the cell. The soluble protein
fragment that is released from the cell contains an epidermal growth factor domain.
Proteasome
Proteases are found in both extracellular (digestive tract, blood, extracellular matix), membrane, adn and intracellular
locations. As mentioned previously, one role of intracellular proteins is to degraded "older" and chemically damaged
proteins. One of the main proteases involved in such intracelluar proteolysis is the large protein complex called
the proteasome. It consist of three large structures
-
one 700,000 MW 20S complex which contains 14 different subunits arranged in 4 rings of 7 subunits (2 copies
of each subunit). The rings are stacked on each other. The outer two rings contain a
subunits while the middle two contain b subunits. ATP-dependent cleavage of protein substrates
occurs within the complex, with N terminal Ser or Thr OH groups acting as nucleophiles.
-
two 19S complex which cap both ends of the 20S complex (not unlike the structure of the E. Coli chaperone complex
GroEL/ES.
Proteins destined for cleavage by the proteasome must first be chemically modified through
attachment of multiple copies of the 8,500 MW protein ubiquitin, a highly conserved protein found ubiquitously in eukaryotes.
The carboxyl group the C-terminal Gly residues forms an amide link to the side chain amine group of Lys
residues in the protein targeted for degradation. The resulting link is an isopeptide bond since the N terminal of the
target protein is not used in the amide bond. Three different proteins are involved in the ubiqutinylation of the target
protein, including E1 (ubiquitin-activating enzyme which requires ATP), E2 (ubiquitin conjugating enzyme) and E3 (ubiquitin-protein
lyase). Once attached, the side chain Lys of ubiquitin can form another isopeptide bond to a C-terminal carboxyl
group of another ubiquitin, forming a growing ubiquitin chain on the target protein. Proteins with 4 or more linked
ubiquitins are better substrates for the proteasome. Proteins with short half-lives (those with certain amino terminal
amino acids like arginine or leucine, or enriched in Pro (P), Glu (E), Ser (S), and Thr (T) - (PEST) appear to be better targets
for the ubiquitin pathway and subsequent degradation by the proteasome.
Proteasome activity is intimately related to health and disease. A major role of
the proteasome occurs in immune recognition of self and nonself. The immune system must be able to recognize a virally
infected or tumor cell (both self cells expressing foreign or aberrant proteins) as well as foreign cells like bacteria, which
can be engulfed by immune cells such as macrophages. Proteasome involvement occurs when viral , tumor , or bacterial
proteins are degraded to short peptides, which bind intercellular major histocompatability proteins (MHC) proteins and are
translocated to the cell membrane. Peptide/MHC complexes are displayed on the cell surface and are recognized by receptors
on immune cells (specifically T cells). Self cells are not recognized by T cells since the peptides in the peptide:MHC
complex are self peptides derived from normal proteins. The T cell receptor recognizes determinants on both the
MHC protein and the presented peptide.
Nonrecognition of self peptide:MHC complexes prevents the immune system from targeting normal
healthy cells. Autoimmune diseases arise when the T cell receptor recognizes presented self peptides. The ubiquitin/proteasome
pathways have been linked to disease manifestation in many neurodegenerative diseases like Alzheimers, Huntington's disease
(which involves the aberrant folding of the Huntington protein which contains an expanded poly-Glu domain), and Parkinsons.
The degradation pathway is involved in many other normal cellular functions including gene transcription and programmed cell
death.
Other Types of Enzymes
The three enzymes studied above are all hydrolases - enzymes that catalyze the hydrolysis
of bonds (either amide or acetal). This is only one class of six different reaction types that have been categorized
by the Enzyme Commission of the International Union of Biochemistry and Molecular Biology. The six types include:
-
EC1: Oxidioreductases - oxdiation/reduction reactions
-
EC2: Transferases - acyl, glycosyl, 1C, N, O, aldehydes, ketones, etc
-
EC3: Hydrolases
-
EC4: Lyases - cleavage of C-C, C-O, C-N, C-S, etc. bonds
-
EC5: Isomerases - racemases, epimerases, cis-trans isomerases
-
EC6: Ligases - form C-C, C-O, C-N, etc bonds
New Ideas on Enzyme Catalysis
Benkovic and Hammes-Schiffer have recently offered an in-depth review of methods used by enzymes
in catalysis. They divided their analysis into a biochemical and theoretical frameworks. From a biochemical viewpoint,
enzyme catalysis was thought to result from binding of substrate followed by activation of the substrate by the enzyme.
The substrate might bind in a particularly reactive conformation in a lock-and-key fashion, or be destorted by the enzyme
on binding, making it more susceptible to bond making/breaking. In an energy diagram for the latter case, the bound
distorted substrate has higher energy than the free substrate, and is an example of substrate (ground state) destabilization.
With the theoretical work of Pauling and development of catalytic antibodies by Lerner, the idea than enzyme functions
by binding the transition state with higher affinity than the substrate became accepted. Evidence for tighter transition
state binding was found for serine proteases (through stabilization of the tetrahedral oxyaninon intermediate) and lysozyme
(through stabilization of the developing oxocarbenium ion (positive charge) by adjacent Asp and Glu residues (both of which
are described above). Quantitative increases in catalysis of phosphoesters and diesters of 1015 to 1017
by some phosphatases and nucleases over the uncatalyzed, spontaneous hydrolysis in aqueous solutions are known.
Assuming that the ratio of the catalyzed rate (kcat/Km with units of M-1s-1) versus the noncatalyzed
rate (pseudo first order with using of s-1) gives a measure of the effective concentration of substrate in the
noncatalyzed rate that would give the same rate as the catalyzed rate, effective concentrations reach as high as 1024
for enzymes like orotic acid decarboxylase. What is the source of this catalytic power? Much evidence has been
accrued to partition catalysis into contributions from general acid/base catalysis, nucleophilic and electrostatic catalysis,
as well as binding effects that enhance catalysis. Solvent effects also show that for some reactions, solvation slows
the reaction, implying desolvation of the substrate before substrate-enzyme binding affects the reaction rate. Remember
that the active site in the E-S complex is devoid of most water molecules that are present in the free enzyme. Finally
the enzyme may have the correct 3D framework that positions all side chains involved in binding in catalysis in optimal positions,
which may have the effect of selecting only catalytically-competent conformations of the substrate (i.e. those the most resemble
the transition state). These contributions suggest that movement of critical residues in the active site is required,
which position the substrate and catalytic residues in optimal positions for catalysis. The kinds of motions (which
can be modeled in molecular dynamics simulations) are described in the table below.
Table: Time Scales of Dynamic Events in Enzyme Catalysis
Motion |
Approx. Time Scale - log(s) |
bond vibration |
-14 to -13 |
proton transfer |
-12 |
hydrogen bonding |
-12 to -11 |
elastic vibration of globular region |
-12 to -11 |
sugar repuckering |
-12 to -9 |
rotation of side chains at surface |
-11 to -10 |
torsional libration of buried group |
-11 to -9 |
hinge bending at domain interfaces |
-11 to -7 |
water structure reorganization |
-8 |
helix breakdown/formation |
-8 to -7 |
allosteric transitions |
-5 to 0 |
local denaturation |
-5 to 1 |
rotation of medium-sized interior sidechains |
-4 to 0 |
from Benkovic, S and Hammes-Schiffer, S. Science. 301, pg 1197 (2003)
Warshel argues that electrostatic stabilization of the transition state is the predominate mechanism for tighter binding of the transition
state than the substrate to the enzyme. Hydrophobic interactions would facilitate proper orientation of the substrate/transition
state in the active site pocket, which is preorganized in effect by the structure of the enzyme. Other have argued that
quantum mechanical tunneling (of hydrides, protons, or electrons) is a main mechanism to surmount energetic barriers in enzyme-catalyzed
reactions. Using theoretical and molecular dynamic simulations, Gao et al argue that tunneling, a way to overcome a
classical energy barrier, plays a much smaller role that reducing the barrier height (activation energy). Warshel notes
that tunneling effects can also occur in solution in the absence of enzyme, making it all the more important to compare reactions
mechanisms in the presence and absence of enzyme. Yet proteins motions that move catalytically important side chains
toward the substrate/transition state may allow both preferential electrostatic stabilization of the transition state and
quantum mechanical tunneling. Yet another idea states that enzymes must create new bonds to substrate/transition state
that could not be available in the absence of the enzyme. One such type of bond is a low-barrier hydrogen bond, in which
a hydrogen on an electronegative atom is involved in strong H bonds to two different hydrogen bond acceptors simultaneously.
Finally, substrate may adopt near-attack conformations (NAC), which more approximate the bound transition state, and it is
the binding of the NAC to the enzyme, not lowering the activation energy, that contributes to the enzyme catalyzed rate enhancement.
|